The inkjet set: 3D bioprinting has travelled far
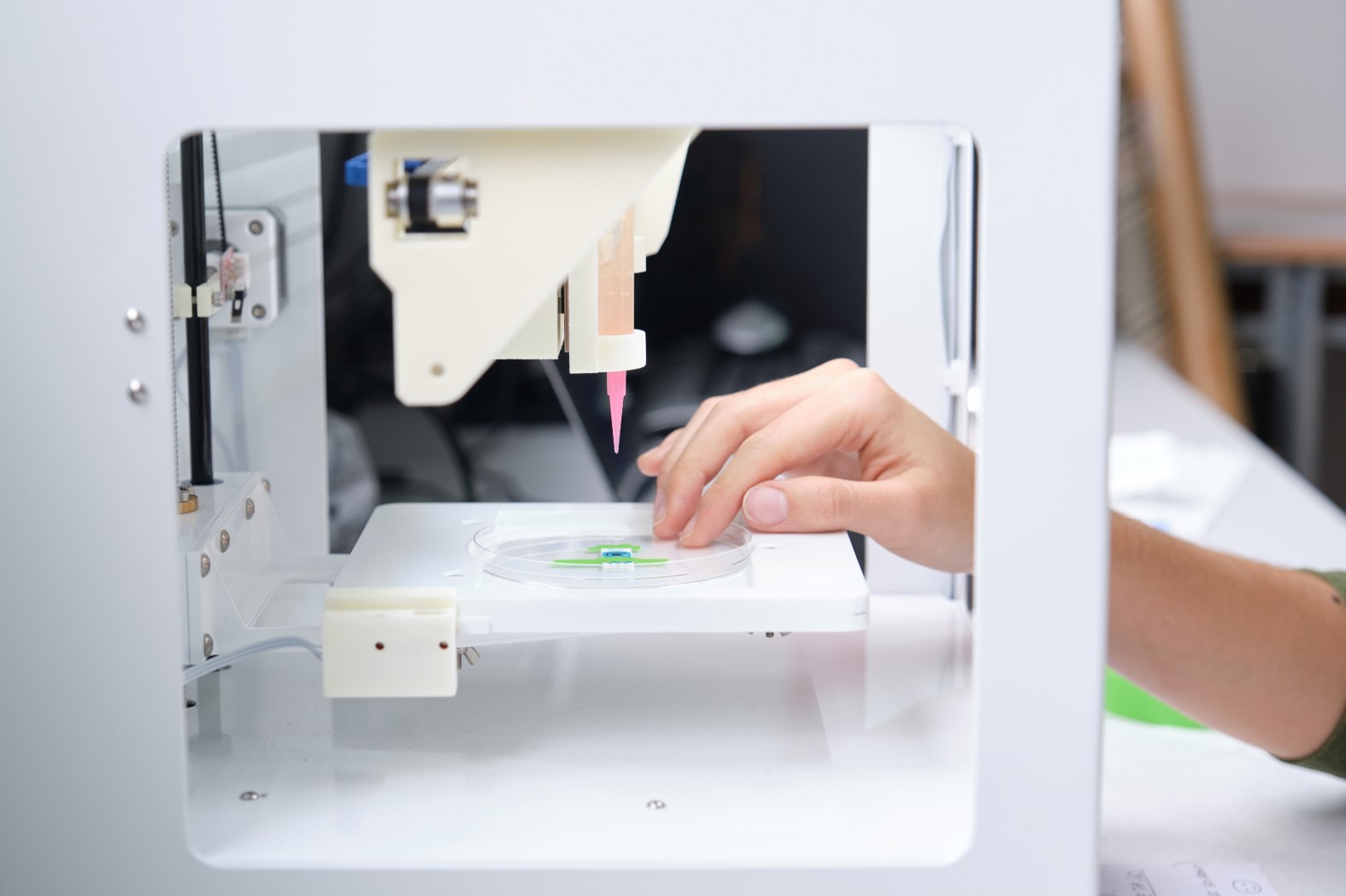
3D bioprinting enables the production of cell-laden models in which cells, biomolecules and biomaterials are deposited in a spatially predefined 3D position. As 3D bioprinting capabilities become more sophisticated, the potential to fabricate functional tissues and organs for drug testing and transplantation is being realized. But with simple stem cell procedures costing $5,000 to $50,000, how many will be able to afford these innovations?
According to Grandview Research, the bioprinting market is currently worth $1.9 billion and is expected to grow to $5.3 billion by 2030 at an average CAGR of 15.3%. This market growth will be powered by technological advances which progress 3D printing through ever more complex lab applications and increasingly widespread adoption in the clinic.
3D bioprinting builds on the technological advances pioneered by 3D printing which transforms 3D computer-aided design (3D-CAD) virtual models into physical 3D objects in a layer-by-layer fabrication technology through the progressive addition of fluid materials (inks) that are polymerized to solids immediately after deposition. The technique provides unprecedented control, flexibility, speed and precision for the direct production of complex elaborate substructures. 3D bioprinting also refers to the printing of materials into shapes for reconstructive surgery. This article focuses on the printing of materials that contains cells.
A critical aspect of 3D bioprinting is the selection of suitable biomaterial inks (bioinks). The bioink is the core component of 3D bioprinting and the synthesis, composition, structure and dynamics of printable bioinks have a great impact on the bioprinting process, and generation of biological structures, especially their mechanical/cellular behaviour. Hundreds of modified biomaterials, primarily hydrogels have been used as bioinks.
The term hydrogel describes 3D viscoelastic polymeric networks, such as agarose or gelatin, formed by crosslinking hydrophilic monomers with covalent/noncovalent interactions. Due to their versatile structure and composition, hydrogels can be engineered using different techniques and/or materials to give them the necessary forms and physicochemical properties, such as porosity, to meet the final product requirements. The vast array of biophysical properties and unique abilities to absorb/retain a significant amount of water allows the engineered hydrogels to replicate the extracellular matrix (ECM) properties of natural tissues.
Hydrogels can be classified as natural or synthetic polymers. Natural hydrogels (alginate, chitosan, collagen, etc.) derived from proteins, polysaccharides and decellularized tissues. These materials can interact favourably in vivo, and possess low toxicity and increased biocompatibility/biodegradability compared to other biomaterials. However, natural hydrogels present some limitations, including weak mechanical properties, high batch-to-batch variability and potential immunogenicity when used in-vivo. In some cases, there is also the potential for transmission of pathogens.
Consequently, over the past decade, natural polymers have been slowly replaced by synthetic alternatives. Although lacking any inherent bioactivity, synthetic polymers (PVA, PEG, etc.) are more versatile and can be designed to yield desired mechanical/physicochemical properties under molecular/macromolecular designs. Novel approaches to constructing these materials have also led to the design of hybrid hydrogel systems that incorporate both natural and synthetic polymers and other functional moieties for better functions.
While a hydrogel's design and material selection are important for function, crosslinking approaches employed to create connections between macromolecules are critical for the final product. Based on their method of preparation, hydrogels are primarily classified as physical, chemical or biochemical. At this stage, their physicochemical parameters and biological performance can also be further tuned/enhanced through various specific processing techniques, crosslinking agents and/or additives.
One unique processing technique is through the formation of multi-layered hydrogels with a spatially-varying matrix composition and mechanical properties by polymerizing the hydrogel matrixes in a layer-by-layer fashion. Another emerging technique takes advantage of freeze-casting and salting-out processes to induce hierarchical morphologies and anisotropic mechanical properties.
Over the last few decades, the development of stimuli-responsive hydrogels capable of adapting to external cues has become a hot topic in the biomedical engineering field. Popularly known as smart hydrogels, these materials are capable of reversibly changing their physicochemical properties, swelling ability, molecule permeability, etc. in response to changes in pH, temperature, light, electric/magnetic fields and the biomolecules’ concentration.
As the utility of a particular hydrogel structure/composition varies over a range of applications, new innovative solutions of bioinks and crosslinking agents are continuously being discovered. Substantial progress has also been made in the design, fabrication, properties and applications of 3D hydrogel construct biomedical engineering. A summary of different hydrogel materials, cell types being used, target applications and the corresponding fabrication methods is presented here.
Despite significant advances, several parameters, including degradation rates, reaction time, surface hybridization, microstructures, immunological response, etc., still demand careful assessment to synthesize cytocompatible 3D hydrogel constructs. Additionally, success in the fabrication of tissue constructs has been largely limited to single component hydrogels, including alginate, cellulose, gelatin, polyacrylates, and hyaluronic acid. Current research is also more focused on altering the chemistry of biopolymers, leading to a significant improvement in the mechanical/biological properties of hydrogels—with a lack of a larger variety of printable hydrogel systems to further advance this field.
In future, on-demand design/development of new materials and/or printing technologies to improve 3D printability and biocompatibility, such as generating vasculature at scale, are still needed to fully unleash the huge potential of 3D bioprinting. At the same time, 4D bioprinting, which uses variable parameters over time during the printing process to alter smart hydrogel characteristics, promises a solution to some challenging obstacles in the fabrication of 3D mimetic scaffolds of appropriate mechanical strength to support cellular viability and printability.
IMAGE: Shutterstock